Chapter 14: Launch
Chapter Objectives
Upon completion of this chapter you will be able to describe the role launch sites play in total launch energy, state the characteristics of various launch vehicles, and list factors contributing to determination of launch windows. You will be able to describe how the launch day of the year and hour of the day affect interplanetary launch energy and list the major factors involved in preparations for launch.
Mission Operations Phases
Interplanetary mission operations may be considered in four phases: the Launch Phase, the Cruise Phase, the Encounter Phase, and, depending on the state of spacecraft health and mission funding, the Extended Operations Phase. These "phases" are actually subcategories of a Flight Project's Phase E, Mission Operations and Data Analysis, as discussed in Chapter 7. Each of these mission operations phases, and subjects pertinent to them, are covered starting in this Chapter, and continuing in the next three Chapters.
Launch Vehicles
The launch of a spacecraft comprises a period of powered flight during which the vehicle rises above Earth's atmosphere and accelerates at least to orbital velocity. Powered flight ends when the rocket's last stage burns out, and the spacecraft separates and continues in freefall. If the spacecraft has achieved escape from Earth's gravitation, rather than entering Earth orbit, its flight path will then be purely a solar orbit of some description (since the launch pad was also in solar orbit).
To date, the only practical way to produce the propulsive energy needed for launching spacecraft from Earth has been by combustion of chemical propellants. Mass drivers, however, may be useful in the future for launching material from the Moon or other small airless bodies. lon-engine propulsion, whether powered by photovoltaics or nuclear reactors, is useful not for launch phase, but for gently but steadily accelerating spacecraft that are already in Earth orbit or beyond.
As first discussed in Chapter 3, there are two groups of propellants for chemical-combustion rockets, liquids and solids. Many spacecraft launches involve the use of both types of rockets, for example the solid rocket boosters attached to liquid-propelled rockets. Hybrid rocket engines use a combination of solid and liquid propellants. Solid rockets are generally simpler than liquid, but they cannot be shut down once ignited. Liquid and hybrid engines may be shut down after ignition and, in some designs, can be re-ignited as needed.
Expendable launch vehicles, ELV, are used once. The U.S. Space Transportation System, STS, or Space Shuttle, was designed as a reuseable system to reach Low Earth Orbit. Most of its components were refurbished and reused multiple times.
Upper Stage Rockets can be selected for placement atop the launch vehicle's lower stages (or within the Shuttle's cargo bay) to provide the performance needed for a particular payload. The Centaur high-energy upper stage has been a choice for robotic missions to the Moon and planets for many years.
A sampling of launch vehicles of interest to interplanetary mission follows. One useful measure of performance for comparison among launch vehicles is the amount of mass it can lift to Geosynchronous Transfer Orbit, GTO.
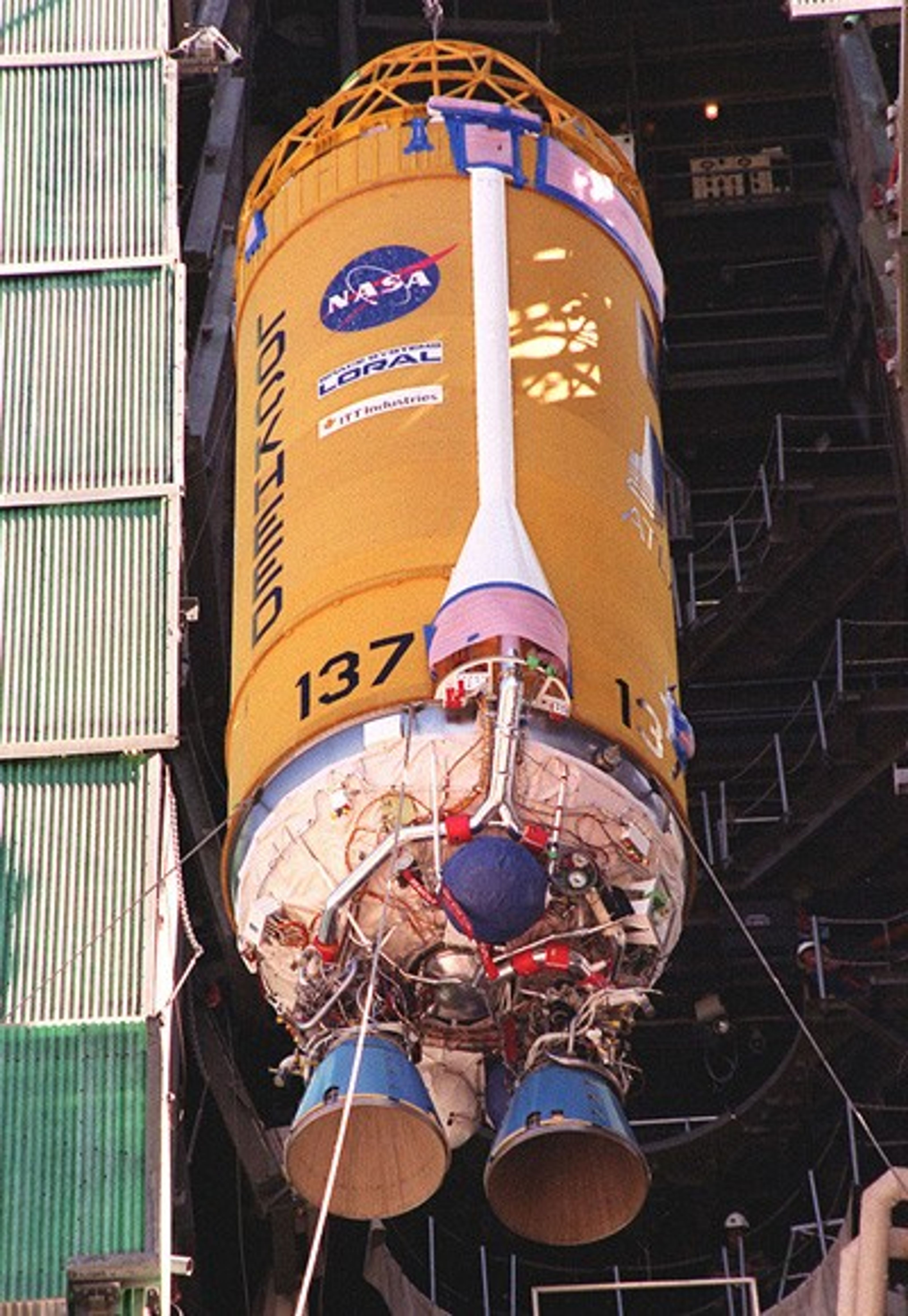
Delta
Delta is a family of two- or three-stage liquid-propelled ELVs that use multiple strap-on solid rocket boosters in several configurations. Originally made by McDonnell Douglas, it is now produced and launched by the Boeing and Lockheed Martin joint venture, United Launch Alliance (ULA).
The Delta II, whose liquid-propellant engines burn kerosene and liquid oxygen (LOX), can be configured as two- or three-stage vehicles and with three, four or nine strap-on solid rocket graphite epoxy motors. Delta II payload delivery options range from about 891 to 2,142 kg to geosynchronous transfer orbit (GTO) and 2.7 to 6.0 metric tons to low-Earth orbit (LEO). Two-stage Delta II rockets typically fly LEO missions, while three-stage Delta II vehicles generally deliver payloads to GTO, or are used for deep-space explorations such as NASA's missions to Mars, a comet or near-Earth asteroids.
The Delta IV family of launch vehicles is capable of carrying payloads ranging from 4,210 kg to 13,130 kg to GTO. The three Delta IV Medium-Plus vehicles use a single common booster core that employs the RS-68 liquid hydrogen/liquid oxygen engine, which produces 2,949 kN (663,000 lb) of thrust. They are augmented by either two or four 1.5-meter diameter solid rocket strap-on graphite epoxy motors.
Delta's launch record (350 flights as of January 2013) includes Earth orbiters and interplanetary missions dating back to 1960. Space Launch Complex 37, a historic Saturn-1 launch pad at the Kennedy Space Center (KSC), has become the site for the Delta-IV launch facility.
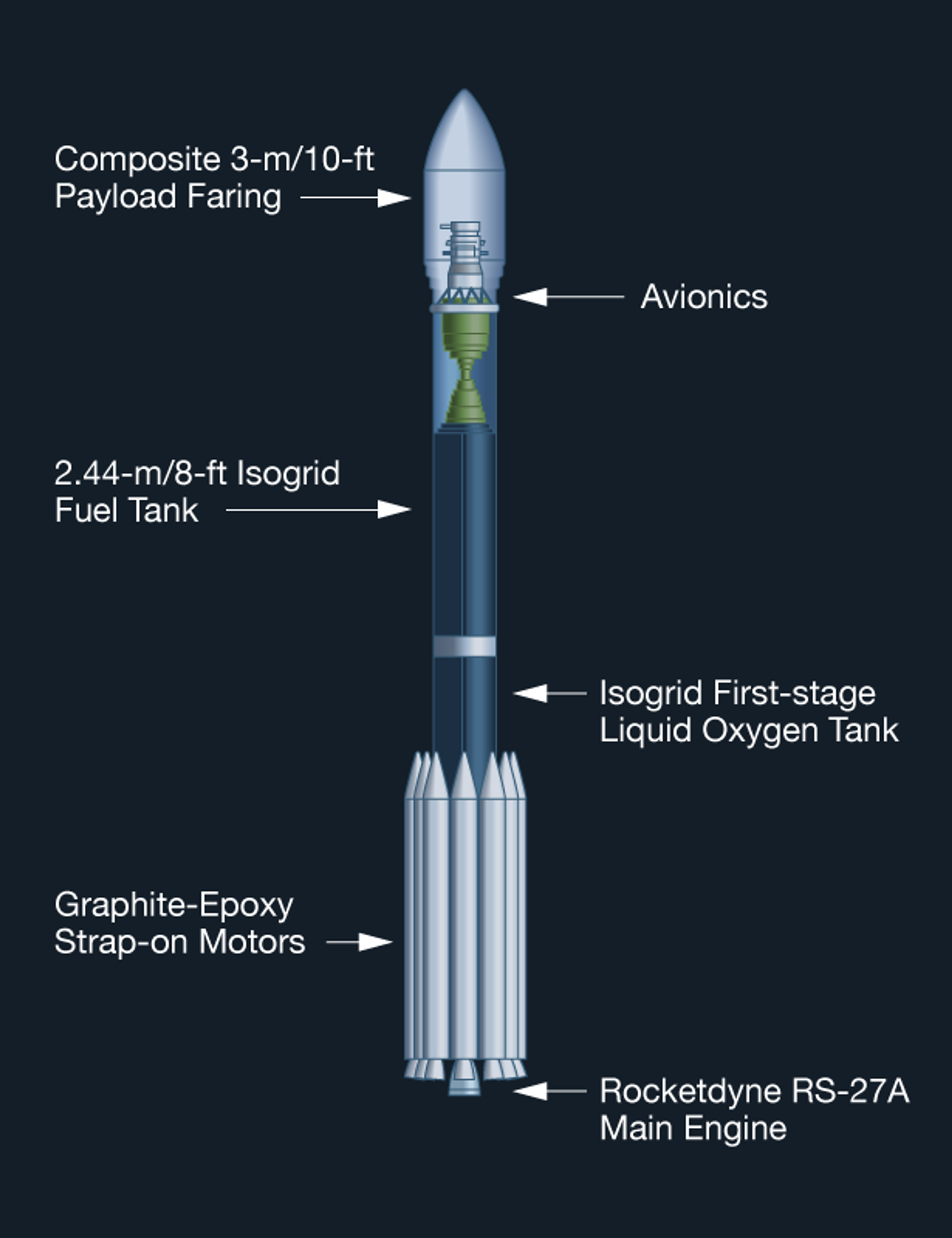
Titan
Titan was a family of U.S. expendable rockets used between 1959 and 2005. A total of 368 rockets of this family were launched. Titan IV, produced and launched for the U.S. Air Force by Lockheed Martin, was the nation's most powerful ELV until it was retired in 2005.
Titan IV was capable of placing 18,000 kg into LEO, over 14,000 kg into polar orbit, or 4,500 kg into a geostationary transfer orbit (GTO).
A Titan III launched the Viking spacecraft to Mars in 1975.
A Titan IV, equipped with two upgraded solid rocket boosters and a Centaur high-energy upper stage, launched the Cassini spacecraft on its gravity-assist trajectory to Saturn in 1997.
Titan III vehicles launched JPL's Voyager 1 and 2 in 1977, and the Mars Observer spacecraft from the Kennedy Space Center (KSC), Cape Canaveral in 1992.
A Titan IV consisted of two solid-propellant stage-zero motors, a liquid propellant 2-stage core and a 16.7-ft diameter payload fairing. Upgraded 3-segment solid rocket motors increased the vehicle's payload capability by approximately 25%. The Titan IV configurations included a cryogenic Centaur upper stage, a solid-propellant Inertial Upper Stage (IUS), or no upper stage. Titan IV rockets were launched from Vandenberg Air Force Base, California, or Cape Canaveral Air Station, Florida.

Atlas
Atlas, the Atlas V rocket is an expendable launch vehicle formerly built by Lockheed Martin. It is now built by the Lockheed Martin-Boeing joint venture United Launch Alliance. Aerojet develops and manufactures the Atlas V boosters.
The rocket, built in Decatur, Alabama, consists of a first stage powered by kerosene and liquid oxygen, which uses a Russian made RD-180 engine, and a liquid hydrogen liquid oxygen powered Centaur upper stage. Some configurations also use strap-on booster rockets.
Together these components are referred to as the Atlas launch vehicle.
The Atlas-IIAS can put 3,833 kg in GTO.
The Atlas-IIA was retired in December 2002, and the Atlas II was retired in March 1998. Re-engineering the Atlas-II booster resulted in an Atlas-III with at least a 5 percent improvement in performance.
The Atlas-V uses a "structurally stable" design; previous Atlas models depended on a pressurized propellant load for structural rigidity. With provisions for adding between one and five strap-on solid rocket boosters, the Atlas-V offers the capability of placing up to 8,670 kg in GTO. View a 2-minute video of the first Atlas-V launch in August 2002.
Space Launch Complex 41 at the Kennedy Space Center (KSC), where Viking-1 and -2, and Voyager-1 and -2 began their journeys, has become the site for the Atlas-V launch facility (see below).

Ariane
Today, Arianespace serves more than half the world's market for spacecraft launches to geostationary transfer orbit (GTO). Created as the first commercial space transportation company in 1980, Arianespace is responsible for the production, operation and marketing of the Ariane 5 launchers.
Ariane 5, launched from the Kourou Space Center in French Guiana, entered service with a 6.5 metric ton payload capability to geostationary orbit.
In 1996, the maiden flight of the Ariane 5 launcher ended in a failure.
In 1997 Ariane 5's second test flight succeeded, and it is now in service.
Proton
The Proton is a liquid-propellant ELV, capable of placing 20,000 kg into LEO, originally developed by the Soviet CIS Interkosmos. It is launched from the Baykonur Kosmodrome in Kazakhstan, with launch services marketed by International Launch Services (a company formed in 1995 by Lockheed Martin, Khrunichev Enterprises and NPO Energia).
With an outstanding reliability record and over 200 launches, the Proton is the largest Russian launch vehicle in operational service. It is used as a three-stage vehicle primarily to launch large space station type payloads into low earth orbit, and in a four-stage configuration to launch spacecraft into GTO and interplanetary trajectories.
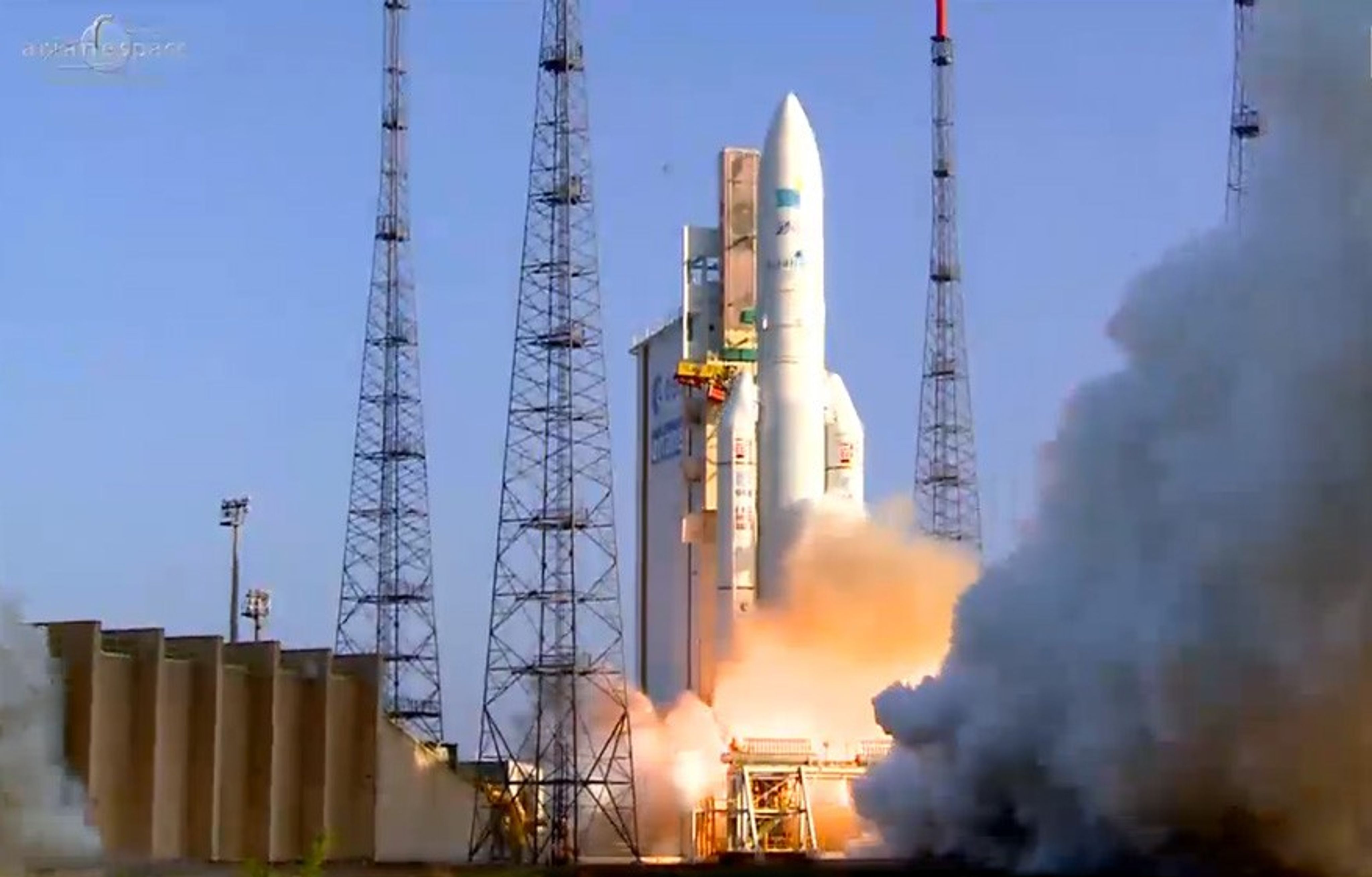
Soyuz
The proven Soyuz launch vehicle is one of the world's most reliable and frequently used launch vehicles.
As of January 2013, almost 1,800 missions have been performed by Soyuz launchers to orbit satellites for telecommunications, Earth observation, weather and scientific missions, as well as for piloted flights.
Soyuz is being evolved to meet commercial market needs, offering payload lift capability of 4,100 kg. to 5,500 kg. into a 450-km. circular orbit. Soyuz is marketed commercially by Starsem, a French-registered company.
The Russian government is directing development of a new launcher under the Prospective Piloted Transport System (PPTS) project.

Space Transportation System
America's Space Shuttle, as the Space Transportation System (STS) is commonly known, was a reusable launching system whose main engines burned liquid hydrogen and LOX.
After each flight, its main components, except the external propellant tank, were refurbished to be used on future flights.
The STS could put payloads of up to 30,000 kg in LEO.
With the appropriate upper stage, spacecraft could be boosted to a geosynchronous orbit or injected into an interplanetary trajectory.
Galileo, Magellan, and Ulysses were launched by the STS, using an Inertial Upper Stage (IUS), which is a two-stage solid-propellant vehicle.
The STS could be operated to transport spacecraft to orbit, perform satellite rescue, assemble and service the International Space Station, and carry out a wide variety of scientific missions ranging from the use of orbiting laboratories to small self-contained experiments.
Thirty years after its first flight, the STS was retired in 2011. Its planned successor, the Space Launch System (SLS), was defined as part of the NASA Authorization Act of 2010 and announced in 2011, and will carry on the duties of the Space Shuttle.
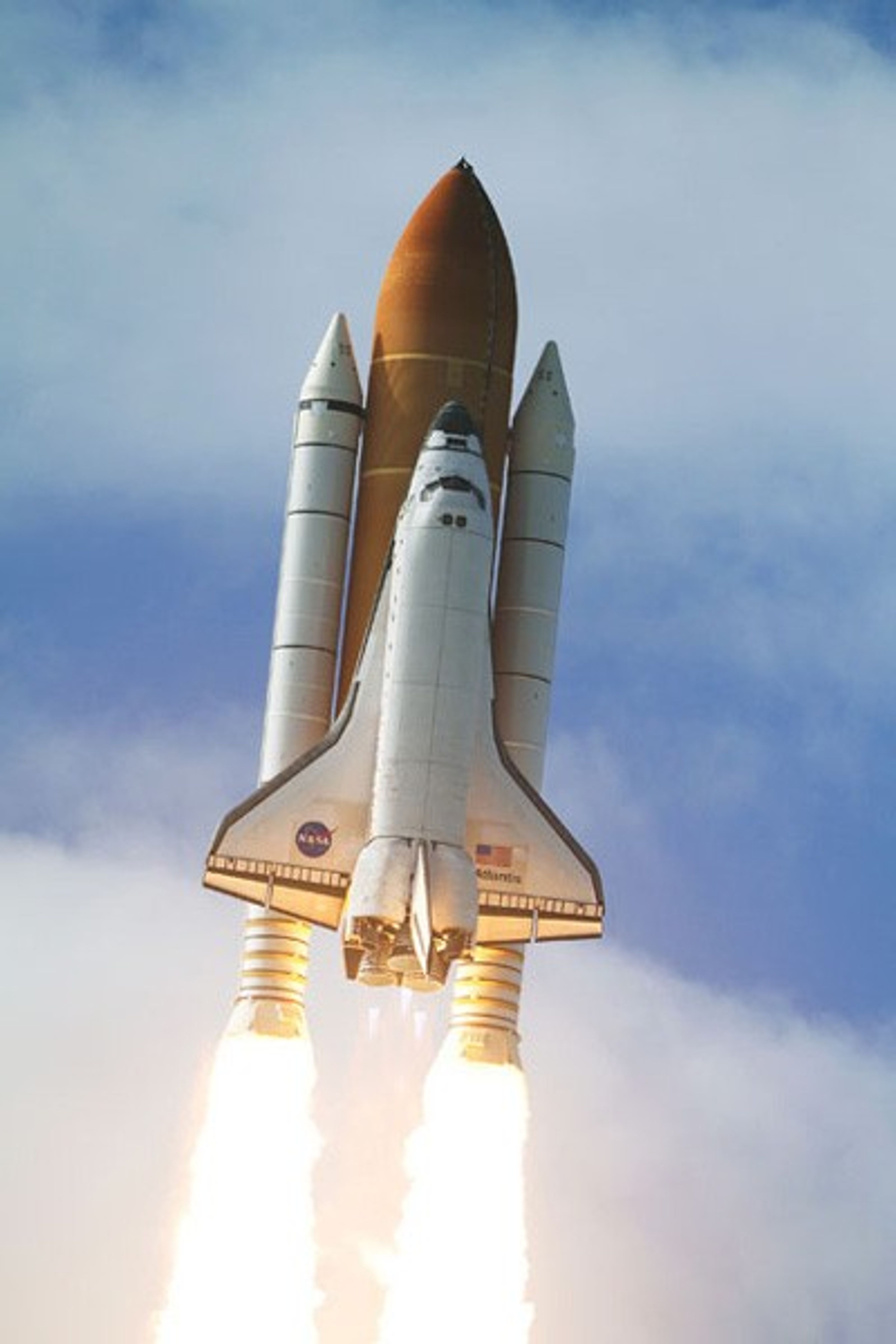
Smaller Launch Vehicles
Many NASA experiments, as well as commercial and military payloads, are becoming smaller and lower in mass, as the art of miniaturization advances. The range of payload mass broadly from 100 to 1300 kg is becoming increasingly significant as smaller spacecraft are designed to have more operational capability. The market for launch vehicles with capacities in this range is growing.
Pegasus is a small, winged solid-propellant ELV built and flown by Orbital Sciences Corporation. It resembles a cruise missile, and is launched from under the fuselage of an aircraft while in flight at high altitude, currently Orbital Sciences' L-1011. Pegasus can lift 400 kg into LEO.
Taurus is the ground-based variant of Orbital Sciences Corporation's air-launched Pegasus rocket. This four-stage, transportable, inertially guided, all solid propellant vehicle is capable of putting 1,350 kg into LEO, or 350 kg in GTO.
Saturn V
The Saturn V launch vehicle served admirably during the Apollo Program that landed a dozen humans on the Moon. Although this vehicle was never used again, honorable mention seems appropriate. This most powerful rocket ever launched was developed at NASA's Marshall Space Flight Center under the direction of Wernher von Braun. Fifteen were built. Its fueled first stage alone weighed more than an entire Space Shuttle.
Perspective: Launch Mass
Contributed by JPL's Kevin Peter Hand, who serves as Deputy Project Scientist, Europa. This is an excerpt from his book "Alien Oceans" (Princeton University Press, 2020):
In the space world we suffer from what many refer to as the "tyranny of the rocket equation." Simply put, what goes up takes fuel — and if fuel is going up, it also takes fuel to lift the fuel. The most cleverly engineered, efficient rocket is still about 85% fuel by mass. Everything else, from the structure of the rocket to the payload sitting atop it (collectively called the "dry mass"), is only 15% of the total mass on the launchpad.
To put this in perspective, consider your car. A gallon of gasoline weighs about 6.2 pounds (2.8 kg), and a typical gas tank holds 12-15 gallons, which is 75-93 pounds of gasoline. If cars were subject to the rocket equation [see Basics/chapter 3-2], then the total mass of your car and anything in it would be limited to, at best, 13-16 pounds. Most cars weigh 3,200-4,200 pounds. The "dry mass" of a car is much more than the "wet mass" of the fuel it carries.
When it comes to going straight up into space, the rocket equation really is a tyrant. The laws of physics are kind to horizontal rolling motion (what a car does), but very unkind to vertical motion against gravity. That is ultimately why the rocket equation is so harsh: you are trying to move directly up and against gravity (or, if landing, trying to protect yourself from gravity and stop yourself from crashing). The rocket equation has forced us in the world of space exploration to always be thinking about ways to make our robotic vehicles and instruments smaller and lighter. It is a frustrating but useful force. We have to tighten our belts and think hard about exactly which measurements we want to make and which instruments to send. The rocket equation inspires creativity and innovation.
Consider what has been done on the surface of Mars. The Curiosity rover on Mars has a mass of just over 1,980 pounds (900 kg), of which 165 pounds (75 kg) are science instruments. The total payload mass, which includes the cruise stage to get to Mars and all the rockets and components needed to land, comes in at 8,463 pounds (3,893 kg). So already we are looking at a ratio of 165 pounds of science instruments to 8,463 pounds of total payload mass. That ratio is 0.019, or just under 2%.
But all of this is riding atop a rocket. How much did that rocket and all its fuel weigh? The Curiosity rover launched on an Atlas V 541 rocket which, when fully fueled and with the payload attached, weighed 117 million pounds (53,000 kg): Let me repeat that with some zeroes in place: 1,170,000 pounds! We landed 165 pounds of science instruments on Mars but it required 1, 169,835 pounds of additional mass to get it there and to make it all work once on the surface. The ratio of instrument mass to total rocket mass is only a bit more than one one-hundredth of a percent of the total mass (0.014%).
Again, returning to the car example, imagine if the "science payload" that you were transporting were groceries, and you were constrained by the rocket equation and the numbers above for getting to Mars. If you had a 15-gallon tank, then the mass of your gas would be about 93 pounds; everything else would need to add up to 16 pounds, which is a total of 109 pounds. Taking 0.014% of that leaves you with only 0.015 pounds of groceries! One average-sized grape weighs about 0.015 pounds. That's what you get to bring home on a full tank of gas! As depressing as this analogy sounds, the brightest rocket scientists in the world would be thrilled to get that little grape back home.
Welcome to the world of spaceflight and the tyranny of the rocket equation. This is the universe in which we live.

Launch Sites
If a spacecraft is launched from a site near Earth's equator, it can take optimum advantage of the Earth's substantial rotational speed. Sitting on the launch pad near the equator, it is already moving at a speed of over 1650 km per hour relative to Earth's center. This can be applied to the speed required to orbit the Earth (approximately 28,000 km per hour).
Compared to a launch far from the equator, the equator-launched vehicle would need less propellant, or a given vehicle can launch a more massive spacecraft.
A spacecraft intended for a high-inclination Earth orbit has no such free ride, though. The launch vehicle must provide a much larger part, or all, of the energy for the spacecraft's orbital speed, depending on the inclination.
For interplanetary launches, the vehicle will have to take advantage of Earth's orbital motion as well, to accommodate the limited energy available from today's launch vehicles. In the launch animation below, the launch vehicle is accelerating generally in the direction of the Earth's orbital motion (in addition to using Earth's rotational speed), which has an average velocity of approximately 100,000 km per hour along its orbital path.

In the case of a spacecraft embarking on a Hohmann interplanetary transfer orbit, recall the Earth's orbital speed represents the speed at aphelion or perihelion of the transfer orbit, and the spacecraft's velocity merely needs to be increased or decreased in the tangential direction to achieve the desired transfer orbit.
The launch site must also have a clear pathway downrange so the launch vehicle will not fly over populated areas, in case of accidents. The STS has the additional constraint of requiring a landing strip with acceptable wind, weather, and lighting conditions near the launch site as well as at landing sites across the Atlantic Ocean, in case an emergency landing must be attempted.
Launches from the east coast of the United States (the Kennedy Space Center at Cape Canaveral, Florida) are suitable only for low inclination orbits because major population centers underlie the trajectory required for high-inclination launches. High-inclination launches are accomplished from Vandenberg Air Force Base on the west coast, in California, where the trajectory for high-inclination orbits avoids population centers. An equatorial site is not preferred for high-inclination orbital launches. They can depart from any latitude.
Complex ground facilities are required for heavy launch vehicles, but smaller vehicles such as the Taurus can use simpler, transportable facilities. The Pegasus requires none once its parent airplane is in flight.
Launch Windows
A launch window is the span of time during which a launch may take place while satisfying the constraints imposed by safety and mission objectives. For an interplanetary launch, the window is constrained typically within a number of weeks by the location of Earth in its orbit around the Sun, in order to permit the vehicle to use Earth's orbital motion for its trajectory, while timing it to arrive at its destination when the target planet is in position. The launch window may also be constrained to a number of hours each day, in order to take best advantage of Earth's rotational motion. In the illustration above, the vehicle is launching from a site near the Earth's terminator which is going into night as the Earth's rotation takes it around away from the Sun. If the example in the illustration were to launch in the early morning hours on the other side of the depicted Earth, it would be launching in a direction opposite Earth's orbital motion.
These examples are over-simplified in that they do not differentiate between launch from Earth's surface and injection into interplanetary trajectory. It is actually the latter that must be timed to occur on the proper side of Earth. Actual launch times must also consider how long the spacecraft needs to remain in low Earth orbit before its upper stage places it on the desired trajectory (this is not shown in the illustration).
The daily launch window may be further constrained by other factors, for example, the STS's emergency landing site constraints. Of course, a launch which is to rendezvous with another vehicle in Earth orbit must time its launch with the orbital motion of that object. This has been the case with the Hubble Space Telescope repair missions executed in December 1993, February 1997 and December 1999.
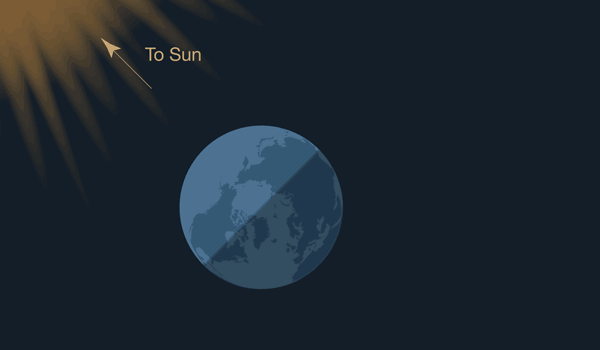
Preparations For Launch
ATLO stands for Assembly, Test, and Launch Operations. This period is usually scheduled very tightly. Spacecraft engineering components and instruments are all delivered according to plan where the spacecraft first takes shape in a large clean room. They are integrated and tested using computer programs for command and telemetry very much like those that will be used in flight. Communications are maintained with the growing spacecraft nearly continuously throughout ATLO.
The spacecraft is transported to an environmental test lab where it is installed on a shaker table and subjected to launch-like vibrations. It is installed in a thermal-vacuum chamber to test its thermal properties, all the while communicating with engineers. Adjustments are made as needed in thermal blanketing, and thermal-vacuum tests may be repeated.
Then the spacecraft is transported to the launch site. The spacecraft is sealed inside an environmentally controlled carrier for the trip, and internal conditions are carefully monitored throughout the journey whether it is by truck or airplane.
Once at the launch site, additional testing takes place. Propellants are loaded aboard. Any pyrotechnic devices are armed. Then the spacecraft is mated to its upper stage, and the stack is hoisted and mated atop the launch vehicle. Clean-room conditions are maintained atop the launch vehicle while the payload shroud (fairing) is put in place.
Pre-launch and launch operations of a JPL spacecraft are typically carried out by personnel at the launch site while in direct communication with persons at the Space Flight Operations Facility at JPL. Additional controllers and engineers at a different location may be involved with the particular upper stage vehicle, for example the Lockheed personnel at Sunnyvale, California, monitoring performance of the inertial upper stage (IUS) or the Centaur upper stage. The spacecraft's telecommunications link is maintained through ground facilities close to the launch pad prior to launch and during launch, linking the spacecraft's telemetry to controllers and engineers at JPL. Command sequences must be loaded aboard the spacecraft, verified, and initiated at the proper time prior to launch. Spacecraft health must be monitored, and the launch process interrupted if any critical tolerances are exceeded.
As soon as the spacecraft is launched, the DSN begins tracking, acquiring the task from the launch-site tracking station, and the mission's cruise phase is set to begin.
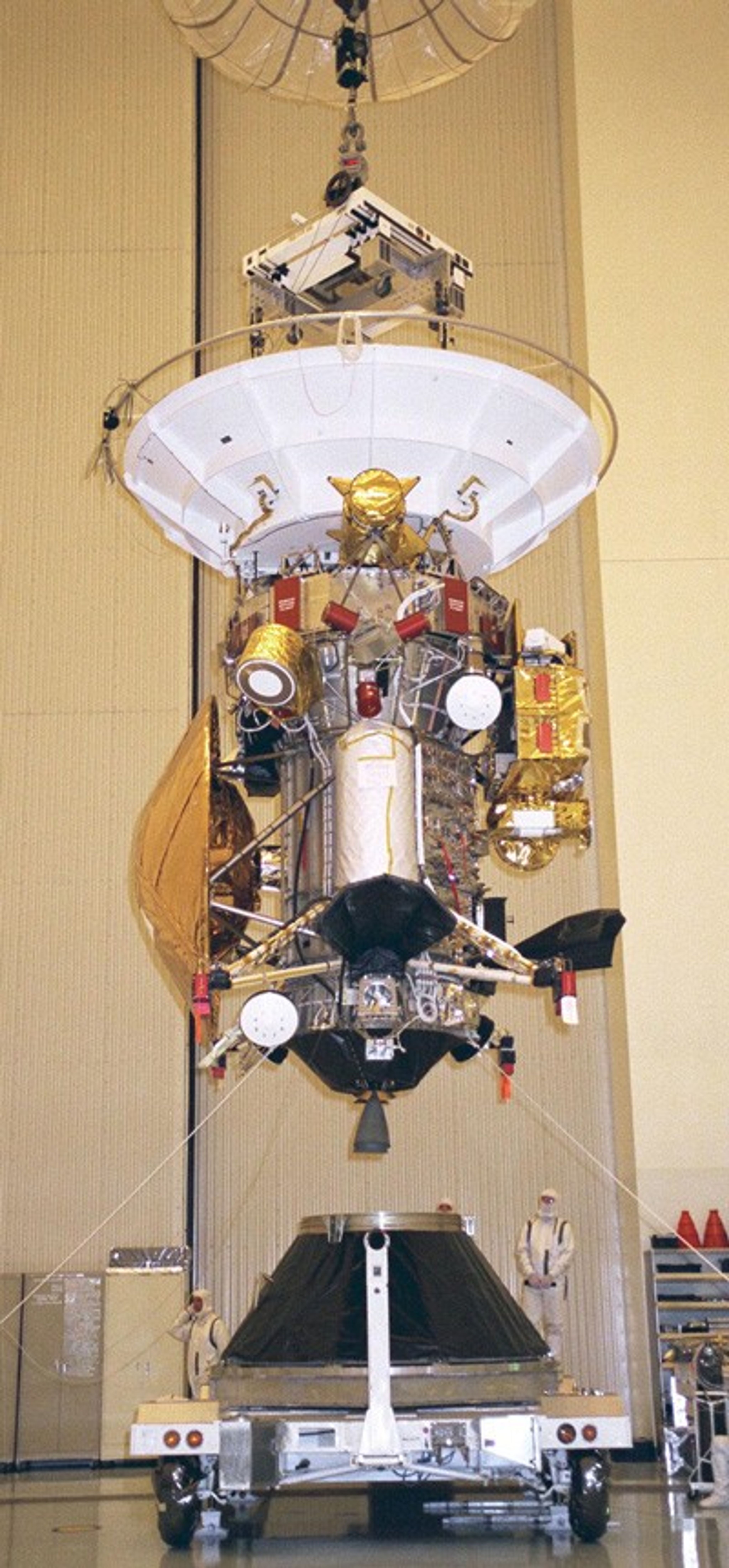
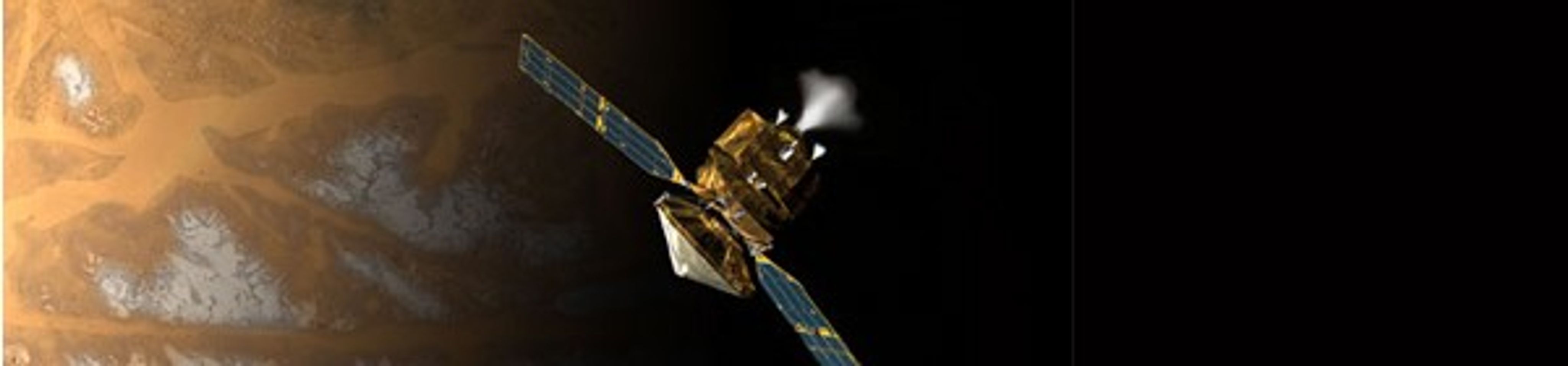
Perspective: A Classic Launch
Contributed by JPL's Arden Acord, who served as the Mars Reconnaissance Orbiter (MRO) Launch Vehicle Liaison for launch in August 2005
Buying Launch
The whole process, from buying the launch service through the actual launch, took about four and a half years for MRO. For most missions that we fly, we don't actually buy a launch vehicle, we buy a "launch service." Kennedy Space Center KSC manages the acquisition process with the support of project personnel. KSC basically has a catalog of launch vehicles that are pre-qualified to fly our class of spacecraft, along with a "not-to-exceed" price for each one. Since there may be more than one rocket type that can perform a mission, KSC may conduct a bid process for each of the launch service providers (e.g., Boeing, Lockheed Martin) to compete for our business. Project people are involved to make sure that all the requirements are right. Once the contract is awarded by NASA, we start the integration process.
Launch Vehicle Integration
There is a long, detailed process of planning how we integrate the spacecraft to the launch vehicle so that everything will work properly. This includes making sure the spacecraft will fit inside the launch vehicle's payload fairing, making sure the spacecraft won't be damaged by the forces put on it during launch, and ensuring that the launch vehicle will put the spacecraft on the right trajectory to get where it needs to go.
The spacecraft is shipped to the launch site at about the same time the launch vehicle is shipped there, three months or so before launch. These two systems go through their final preparations for physical integration. The spacecraft is attached to the launch vehicle adapter, and is then encapsulated in the fairing. The encapsulated spacecraft is transported to the launch pad and is hoisted by crane and attached to the top of the launch vehicle. This happens about 10 days before launch, at which time when the countdown clock typically starts.
The countdown helps everyone orchestrate the many parallel operations that are going on to get everything ready. People have to work at very specific times during the countdown so they don't interfere with one other. During this period, any final operations on the spacecraft take place, including removal of instrument covers and other "remove before flight" items, installation of arming plugs, and generally getting everything buttoned up for the big day.
Launch Day
Around one day before launch, things start to get exciting. The launch vehicle begins its fueling process and final preparations. The spacecraft launch crews come in to power up the spacecraft, load software and send commands that put the spacecraft into its launch configuration. At various times, there are "polls" where the launch managers (including the project manager) report whether they are "go for launch." About five or 10 minutes before launch, the spacecraft is in its launch state, and the launch vehicle goes through its final countdown. During these final minutes, the launch process is controlled mostly by computers. At "T minus zero," if there are no aborts, the rocket with our spacecraft on top begins its journey of discovery.
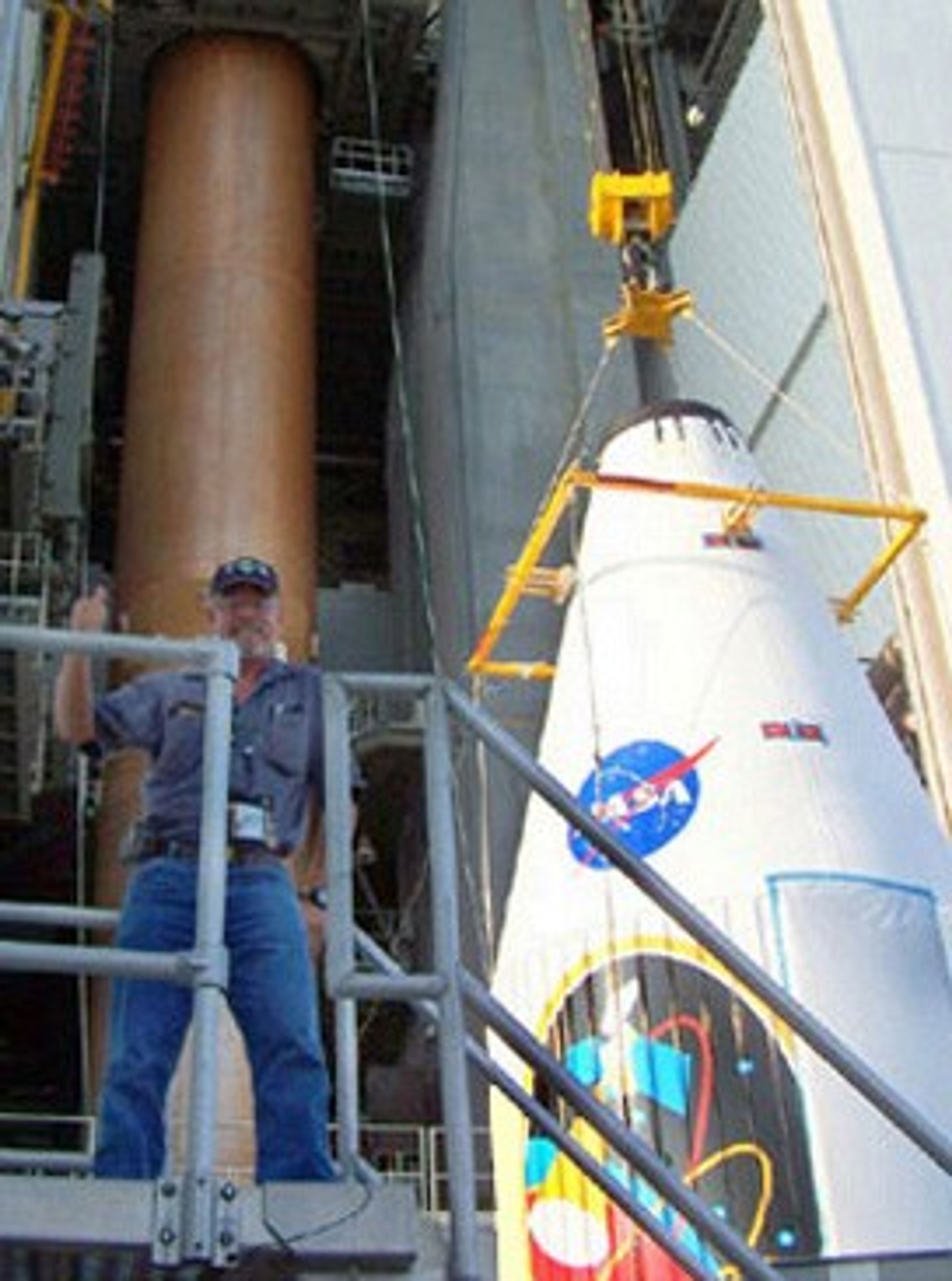